"A cosmic mystery of immense proportions, once seemingly on the verge of solution, has deepened and left astronomers and astrophysicists more baffled than ever. The crux ... is that the vast majority of the mass of the universe seems to be missing." -William J. Broad
It was in the 1930s, looking at dense clusters of galaxies (like Coma, below), that Fritz Zwicky first noticed that the mass in the Universe didn't add up.
We knew how gravity worked, so it was pretty straightforward -- based on how the galaxies within the cluster were moving -- to calculate how much the total mass of this cluster was.
It was also straightforward, because we knew how stars worked, too, to calculate how much mass was in all the stars in all the galaxies that made up the cluster. All you had to do was measure the starlight, and there you had it: two different ways to measure the mass of the same set of objects.
At least, you'd expect these two numbers to match up, if the stars were what made up the mass in the Universe. It turns out not to be the case, and it turns out not to be close: the two numbers you get for mass -- the one from gravity and the one from starlight -- differ by a factor of fifty.
"That's okay," you say. Because there's ever so much more than stars out there.
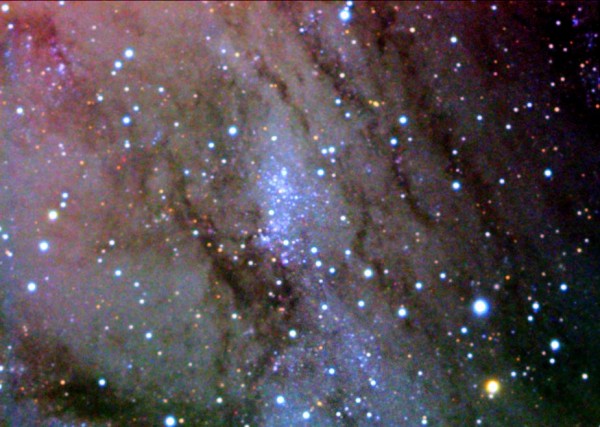
Matter not only clumps and clusters into stars, but also planets, gas, dust, plasma, asteroids, comets, ice, and -- every once-in-a-while -- living creatures. So you could imagine that this "missing mass" out there, the other 98%, was some other form of protons, neutrons, and electrons like the stars are.
We actually have three completely independent ways to measure, very accurately, just how much of the Universe is made out of this "normal stuff" that we're familiar with.
We can trace back the physics of the Early Universe to the first few minutes after the Big Bang, and calculate how much of the light elements -- hydrogen, helium, lithium and their isotopes -- should have been created during this period of Big Bang Nucleosynthesis (BBN). It's dependent on one parameter and one parameter only: the ratio of number-of-baryons (protons and neutrons combined) to photons (which we can count). So we measure the light elements and we get a number for how many protons and neutrons (and since the Universe is neutral, we know electrons, too) there are in the Universe.
We can look at the pattern of fluctuations in the Cosmic Microwave Background (CMB). The positions and heights of the different peaks tell us two things: how much normal matter (protons, neutrons and electrons) there is in the Universe, as well as how much total matter (all things with a gravitational mass, combined) there is. Again, we get a number.
And finally, we can look at the Universe on the largest scales. We can observe how galaxies, clusters and superclusters of galaxies group together, and probe the Universe's Large Scale Structure (LSS). The amplitude of those wiggles -- as well as the overall height of this graph (the power) -- gives us a different way of measuring those same two things: the amount of normal matter and the amount of total matter.
What we find is in remarkable agreement. All three measurements (BBN, CMB, and LSS) all give the same values: about 15% of the total amount of matter -- including the stars -- is normal matter, and the rest of it, some 85%, is matter that doesn't emit or absorb light. Throw in the fact that all of this matter combined is just over 30% of the total energy in the Universe (with the rest being dark energy), and we find that less than 5% of the Universe is made up of normal matter, using three independent measurements.
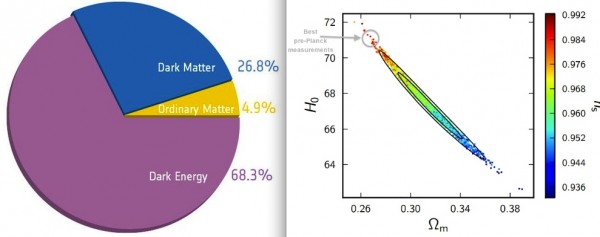
So what is that dark matter? What is that missing mass that doesn't interact with light?
Believe it or not, there's a candidate particle -- the second most abundant type of known particle in the Universe -- that comes to us directly, leftover from the Big Bang: the neutrino!
Particle-antiparticle pairs were all created in the early Universe indiscriminately and in great abundance, and there was a time when temperatures and densities were so high that even the humble neutrino (and the antineutrino) were created in that same great abundance.
But when the Universe was very young -- about a single second after the Big Bang -- neutrinos froze out, which means that temperatures and densities dropped enough so that they stopped interacting with other forms of matter and with themselves. If neutrinos had been completely massless, they would have had an energy spectrum and distribution very similar to what leftover photons from the Big Bang -- the Cosmic Microwave Background -- has today.
There would be slight differences: the neutrinos would be at a slightly lower temperature (1.96 K), they would have had only about 2/3 the total energy of the photons (due to both temperature and particle statistics differences), and there would be just under 300 of them permeating every cubic centimeter of the Universe.
But that's assuming neutrinos were massless; if they had a mass -- even if that mass were tiny compared to the other known particles -- the expansion and cooling of the Universe could have left these cosmic neutrinos, outnumbering protons by more than a billion-to-one, as the primary mass source of the Universe!
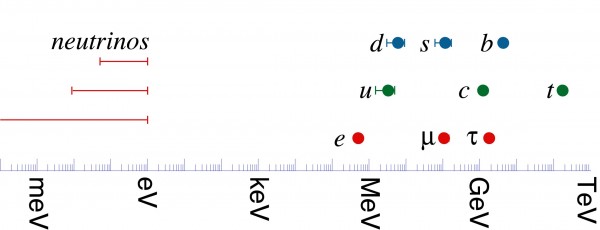
If the neutrinos -- and remember, there are three different types, all in equal abundance to one another -- had had a mass of just 3.7 eV apiece (and remember, the next lightest particle, the electron, has a mass of about 511,000 eV), then 100% of the dark matter would have been accounted for by neutrinos. In fact, it wouldn't have mattered how it was distributed; so long as the masses of the three types of neutrinos (e, μ, and τ) had summed up to 11.2 eV, they would have been all of the dark matter.
So a little bit could have gone a really, really long way!
But even the most beautiful theory, at the end of the day, has to contend with the Universe as it actually exists. From measurements of the cosmic microwave background, where the strongest constraint comes from, we find that the upper limit on the sum of the masses of the three types of neutrino is only 0.18 eV, which means that a maximum of 1.6% of the dark matter is in the form of neutrinos.
And from observations of neutrino oscillations, we know that there's a lower limit on the sums of the neutrino masses: 0.06 eV, meaning that at least 0.55% of the dark matter is in the form of neutrinos.
Neutrinos were moving quickly when the Universe was younger, which means it's a form of hot dark matter. Dark matter that was moving slowly when the Universe was younger was colder, and different structures form on different scales in the Universe dependent on whether the dark matter is hot, warm or cold.
While large-scale structure tells us that the vast majority of the dark matter must be either cold or warm, we know that there is a tiny bit of hot dark matter, and that's in fact what the neutrinos are! So while the large scale structure in the Universe agrees (within the measurable errors) with cold dark matter (CDM, in the figure below), we know that there's a tiny mix -- between 0.55% and 1.6% -- of Hot Dark Matter, in the form of neutrinos, thrown in there!
Now getting the other 98.4-to-99.45% has proved elusive so far, but neutrinos -- the only form of dark matter beyond baryons that we know of -- are not only the tip of the dark matter iceberg, they're actually the only bit of dark matter we know and understand today! Looking at all the matter in the Universe, here's how it's made up:
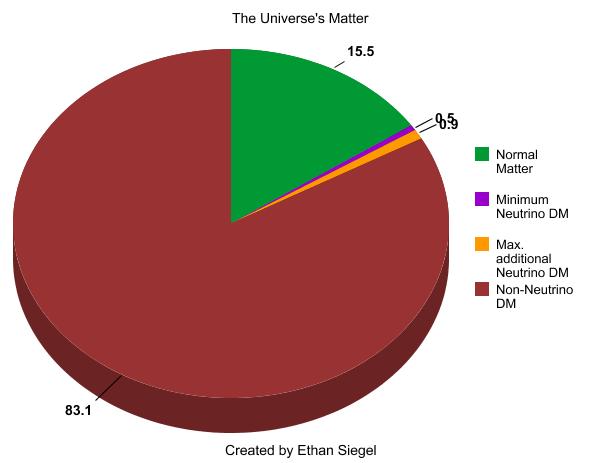
About 15.5% is normal matter: stuff made out of protons, neutrons, and electrons in all their different forms.
Between 0.5% and 1.4%, of all the total matter, is neutrinos: the particles with the smallest non-zero mass known in the Universe, outnumbering protons, neutrons and electrons by more than a billion to one.
And, alas, about 83% of the Universe is some "other" form of dark matter, which must either be cold or warm (not hot), and which we still haven't found.
And that's the story of the little bit of dark matter that we know!
- Log in to post comments
Ooh, there's a lower bound on neutrino mass now. I'd heard that neutrino oscillations implied non-zero mass, but an actual constraint is news to me. Did that come from the recent T2K results?
I love how in such a seemingly short amount of time science can go from "a thing exists" to "here are the parameters of that thing".
Amazing story, well told. I did not know that neutrinos were ever such a strong candidate to explain dark matter.
But as you says, "But even the most beautiful theory, at the end of the day, has to contend with the Universe as it actually exists."
And there we have it, the universe tells us that it doesn't work that way. OKThen.
Thank you Ethan for that humbling piece of science history.
It really was a beautiful theory; let's all applaud the scientists who figured it out. Great sceince.
Moment of bliss.
Now let's all applaud the scientists who figured out why it was wrong!!! Excellent science.
Note page 185 of the Foundation of the General Theory of Relativity where Einstein says "the energy of the gravitational field shall act gravitatively in the same way as any other form of energy". This energy has a mass-equivalence. The neutrino energy has a mass-equivalence too, regardless of the neutrino mass. It's the same for the photon. A massless photon has an "active gravitational mass".
If neutrinos could be a candidate for dark matter, but it turns out that they cannot account for all of it, then is it not possible that the CDM could interact via the weak force? If so, would it be possible to try to set up detectors similar to those used to detect neutrinos? Of course, I realize that detection of particles interacting only via the weak force is difficult, but would such an experiment be possible? Of course, it's entirely possible that the remaining dark matter does not interact via the weak force either, so such tests might turn out to be futile. Also, in such an experiment would it be even possible to distinguish neutrino interactions from those with the cold dark matter particles (whatever they turn out to be), assuming that the weak interaction does exist?
BTW, my last sentence above is badly worded. Obviously, I know that the weak interaction exists. I meant to say ...assuming that the cold dark matter particles will interact with normal matter via the weak interaction?
Sean T:
A good idea! Not only is it possible, there are already a variety of experiments that have been and are being run based on the premise that CDM interacts via the weak force. I don't think there's any way to distinguish directly that an event came from a neutrino, CDM particle, or other source, but by comparing the number and energy distribution of the events to models without CDM they can get an indication that what they are seeing is "new".
The big problem is that as hard as it is to detect neutrinos (at a level that rises above background noise even in heavily shielded underground experiments), it's even harder to detect CDM, especially since now even the more-common neutrino interactions are noise.
Here's a link to one experiment I've been following over the years, CDMS-II, and their latest results:
http://cdms.berkeley.edu/press.html
Here they report that they had *3 whole events* from a run of their detectors that lasted over a year. It deviates from the expectation of the CDM-less model by 3-sigma once you account for the energy distribution. But 3-sigma signals pop up and go away all the time, especially ones based on such tiny amounts of events. They had a previous detection of candidate events from their germanium detectors that was roughly at the same level, but didn't pan out and was eventually ruled to be noise in the electronics.
So they're checking. The nice thing is that even if they never detect a DM particle, simply by checking they are putting constraints on the interaction cross section. If it's zero, then these experiments are going to help us figure that out!
@Sean T #5 and CB #6: Thanks for the CDMS shout-out :-) Technically, we (I'm in the SuperCDMS collaboration) do not assume that DM interacts weakly. In fact, all of the DM non-observation essentially rule that out, since the weak-interaction cross section is easily computed.
What we do assume, because without it we don't have an experiment :-), is that DM can interact _somehow_ with atomic nuclei, causing them to recoil and produce a signal (phonons) in our detectors.
An interesting side note, which comes out in the latest Snowmass report on the future of particle physics: as we move up to ton-scale and larger DM detectors, the irreducable background becomes neutrinos! If the DM-nucleus cross-section is too small, then at some point the rate of neutrinos causing recoils becomes larger than that of DM, and we're screwed :-/
Michael Kelsey: Anytime! Or rather all the time, or rather pretty much every time Dark Matter comes up. :)
I thought I read years ago a paper saying they were using a model of "weak interactions" at the "electroweak scale" -- is this something that was ruled out in the last few years, or did I just misunderstand from the beginning?
Is there another known interaction that isn't ruled out, or is detecting a DM particle going to also mean we've detected a new force?
And lastly, can you give me any hints on what SuperCDMS is up to now? More silicon detector runs in hopes of more events? Upgrades? More processing of existing data to better establish significance? Discovery of WIMPs? Love you guys, but it's hard to wait 4 years between announcements. :)
@CB #8: I can't talk about future plans which haven't been presented (for obvious reasons). Since SuperCDMS is still in the design stage, we're definitely exploring the relative costs and benefits of new silicon detectors, along with the 100 mm Ge we already have prototyped.
One of the beauties of any large experiment is that as people come up with new ideas for analysis or filtering, you can apply those ideas to existing data, without having to wait for future runs. I'm confident we will have several new analyses out before SuperCDMS comes online.
The question of weak vs. otherwise is probably one of terminology. What we assume about dark matter is that the interaction in our detector is basically a coherent elastic collision with the nucleus. We can be agnostic about the underlying force involved, and quote results (or limits) in terms of cross-section vs. DM particle mass.
If the interaction is due to the weak force (Z0 exchange), then the strength would scale with whatever "weak hypercharge" is assigned to the DM particle, and that's an experimental parameter.
Michael: What if I said "pleeeease?" and "come oooooon!" I'll even go as far as "I'll be your best friend!" if necessary. Fair warning. :)
Can you tell me if the existing CDMS-II detectors (I just took SuperCDMS off the project page and didn't understand it's a new thing) are still running?
The prospect of new analysis of the data is exciting on its own. But new data is even better. New data from new detectors better still. :)
Thanks for the explanation of the interaction assumptions.
The original CDMS-II detectors are not running. What we're running now at Soudan is "SCDMS", which is testing new readout masks which we plan to use on the SuperCDMS detectors. They should give better position and energy resolution for both charge and phonon signals.
Probably doesn't work like this, but what if enough neutrinos were moving *obscenely* close to c (as in, enough to raise their mass by the reqd amount)?
A question about CDMS. Aren't they looking for WIMPS as DM candidates? And aren't WIMPS SuperSym. particles?
My questions is: if they turn out to be wimps, how do we reconcile this super symmetry with LHC showing no signs of any super symmetry?
"In fact, all of the DM non-observation essentially rule that out, since the weak-interaction cross section is easily computed."
Doesn't the calculation depend on assuming the weak interaction is based on everything we assume it is from our quite difficult studies of neutrinos?
If the interaction depends on velocity (currently not included, since all neutrinos go so damn fast there's not a lot of difference between their speeds from our frame of reference), then heavy CDM interacting weakly could be much different in cross section.
My understanding of this postgrad-level elementary particle physics is, however, both old and unreliable, so it may be that the inclusion of velocity-of-frame could not be included without breaking some symmetry or summat.
Moreover, the required characteristics of the weak force carriers may be much better known than I've been told and never updated my knowledge and preclude that scenario.
Mark, I believe that the energy of neutrinos already include that, since their rest mass pretty much precludes any appreciable difference between their relativistic mass and their mass as recorded by us. Therefore the same. Capacity for change is already included by our original belief that neutrinos were massless, moving at light speed, and therefore had the same mass in every frame of reference, including one at our velocity (definitionally zero).
Michael: Thanks again!
Sinisa: They're looking for WIMPs, but WIMPs aren't necessarily SUSY particles. SUSY is just a handy theory that predicts the existence of some that would be good DM candidates if in the right energy range. Their search is much more general than just that, and as Michael clarified not even assuming interactions of a type that the SUSY DM candidates would undego.
It could be that CDMS or other searches discover a new WIMPy particle that wasn't predicted by *any* theory. It's been a while since that happened! The theorists would certainly have to take into account the particle not showing up at the LHC, assuming it never does.
@ CB
Thanx for clarification. Appreciated. :)
@Mark: as far as I know neutrinos can only move at c, but relic neutrinos cannot account for the required dark matter.
However note that space has its vacuum energy, and that this has a mass equivalence. Yes, when the energy density is uniform, it has no gravitational effect. But space expands between the galaxies but not within, a thousandfold since the CMBR arose. Conservation of energy would then suggest that spatial energy is not then homogeneous. Every galaxy must surely have its halo of inhomogeneous vacuum. Google on inhomogeneous vacuum. That's what curved spacetime is.
Wow,
Are you saying it's already accounted for? I thought there was no limit to potential relativistic mass though? You can always pump in more energy and add another 9 to the decimal place.
I'm thinking of the famous OMG particle, which apparently had the energy of a tennis ball doing 60mph (!). Sure, it was way more massive than a neutrino to begin with, but if a few percent of neutrinos are moving at a similar pace then each one is worth trillions of slower ones.
(Not sure how they could be accelerated though. Black hole jets?)
John,
I thought they had non-zero mass? Can't hit c?
(Clarification: I'm sure it IS accounted for. Just not sure how.)
Michael Kelsey #7,
Let me ask or ramble about thoughts speculations that your last paragraph triggers.
MK #7, last paragraph
"An interesting side note, which comes out in the latest Snowmass report on the future of particle physics: as we move up to ton-scale and larger DM detectors, the irreducable background becomes neutrinos! If the DM-nucleus cross-section is too small, then at some point the rate of neutrinos causing recoils becomes larger than that of DM, and we’re screwed :-/"
It would seem to me that if neutrinos are an irreducible background; then that means that DM interaction with normal matter is omnidirectional. And in my mind if something locally omnidirectional (i.e. DM) is effecting the 3-D organization of normal matter; then that something may be an interaction from a higher dimension. In the sense that the thumping on a 2-D table top that causes dust or dirt on the top to organize in piles, that that thumping comes from a higher dimension (e.g. outside and orthogonal to the 2-D tabletop). So being experimentally screwed (i.e. not being able to distinquish the omnidirectional influence of dark matter from the omnipresence of neutrinos) would seem to suggest a theoretical possible reason for introducing higher dimensions.
OK that's my first ramble. My question Michael is; am I making any sense?
Regarding the idea that "the DM-nucleus cross-section is too small"; why do we make the assumption that the cross section may be too small? I mean why can't the experimental difficulty be that the cross section of interaction is effectively extremely large (i.e. nonlocal); in the sense that the thumping on a @-D table top would be percieved by the 2-D creatures (on a plie of dust) as a locally unobservable phenomenon (not because the cross section of interaction is too small) but because the cross section of interaction is effectively a very large part of the entire 2-D space. I mean why do we have to assume that a QM state change (associated with DM observations) must be due to a locally observable phenomenon; unless of course we've already assume that it must be contained (i.e. come from) our 3D universe? But why must it? Why not take DM (and DE) as suggestive evidence for extra dimensions.
These questions show my bias that DM (and also DE) missing mass and energy may be violations of conservation of energy due to lack of taking into account energy contributions from extra dimensions. Thus the assumption that our 3-D universe is a closed system is incorrect; our universe may not be closed in the way we think. But I'm asking for experimental observational reasoning thatsupports or refutes my questions (or also, why my questions don't make sense).
It's exhausting; but I expect to personally be attack. I don't learn any physics from that; I do learn if my faulty physical reasoning is pointed out in words that I can understand.
Oh well, my intention is not to provoke; but to learn by listening to any reasoned comments. thank you.
By the way Michael thank you for mentioning Snowmass report. I can't find the report but what I find about the Snowmass particle physics meeting is both readable and very interesting.
"Are you saying it’s already accounted for? I thought there was no limit to potential relativistic mass though? You can always pump in more energy and add another 9 to the decimal place."
The processes that create the neutrinos are, from what I know of the mechanisms, limited in much the same way X rays are merely Gamma rays that are produced by different processes that in the case of Gammas are not limited to the binding energy of the first electron deep inside the atom.
There's a practical limit, therefore, to the energy available to the neutrino in creation (at least for the such-a-vast-majority) that they're limited to the excess energy in the bare creation mechanism. And that puts a limit on the energies, therefore velocity since they're so light it's pretty much identical to c for all of them.
"I’m thinking of the famous OMG particle, which apparently had the energy of a tennis ball doing 60mph (!). Sure, it was way more massive than a neutrino to begin with,"
It was also possible to accelerate. And far easier to spot. Both are the consequence of "it was way more massive than a neutrino to begin with".
Just had to quickly google it because although I've heard of it, it was to me an interesting, but not ground-breaking thing and it was charged, therefore interacts with something other than the weak interaction.
Sorry, could have done this appropriately in the earlier comment:
"John,
I thought they had non-zero mass? Can’t hit c?"
They're extremely light, therefore the difference for any energies they can be left with after creation means they go so fast they're near indistinguishable from c. Indeed that was the reason for them to be considered massless particles: they appeared to be going at c.
There were more reasons than that, but it was a biggee.
Appreciated, thanks.
Mark: the important point is that the mass doesn't matter, it's the energy that matters. A massless photon has a gravitational field. This is so slight as to be undetectable, but if you had a star-sized gendanken* mirror-box containing a zillion gamma photons, you'd be able to detect it easily. It's the same kind of thing for neutrinos. Their mass doesn't matter, their energy is what matters.
* The gedanken mirror-box is considered to have no mass of its own.
Sorry to come in so late on this conversation but I have to address on the one hand the impenetrable wall of denial concerning dark matter and on the other, the plethora of increasingly complex explanations for the invisibility of it and the failure to ‘see’ it. To face down the combined intellectual might of the scientific world is somewhat intimidating and daunting but here goes:
Doug Lowe’s Law states that when an object maintains a stable orbit around another object, the force of gravity between the objects is counter balanced by a propulsive force generated by the orbiting object at right angles to the force of gravity in the direction of motion of the orbiting object.
If you accept this Hypothesis, you have found dark energy and you have no need of dark matter. Dark matter is dead!
What if the big bang created more types of matter than we've encountered. After all, the only matter we can encounter is matter and photons produced by the sun. But the sun isn't even the biggest star out there, and the supernova that created our solar system probably wasn't the biggest form there was out there, either. Our sun isn't even massive enough to produce the heavier elements in our solarsystem, that's why the supernova theory was created. So, theoretically, a larger mass and explosion could result in even heavier elements. The big bang was all of the matter in the universe exploding--that means there pretty much had to have been other elements created. And we can't see them because we haven't evolved to detect the kind of photons they emit, and we don't have any technology to detect it either.